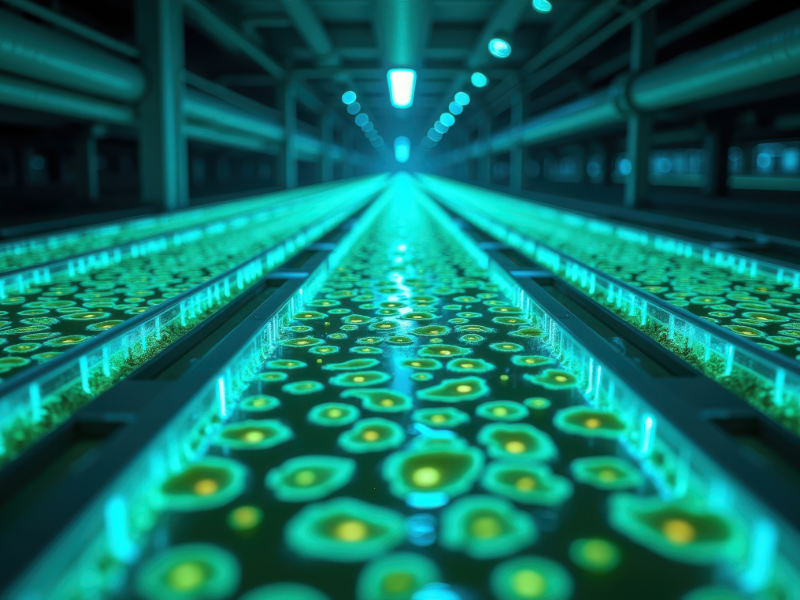
When faced with solving the climate crisis, no idea is too ambitious for Synthetic Biology (SynBio) scientists. While SynBio has already underpinned a range of innovative climate-saving technologies (including biofuels, carbon capture, and many more), a particularly futuristic concept is currently being brought to life: creating new organisms that feed on carbon.
Living organisms can essentially be divided into two groups: autotrophs and heterotrophs. Autotrophs generate nutrients from abiotic sources such as CO2 and store it in organic compounds; heterotrophs get their nutrients by consuming said organic compounds.
Many kinds of autotroph exist, with the most familiar being plants. The supercharging of existing autotrophs is already firmly within SynBio’s purview, but now scientists are broadening their horizons by attempting to engineer entirely new autotrophic organisms that can feed on carbon direct from the air.
The first step in this process is choosing a template to start from. Bacteria, in particular E. coli, have long dominated the genetic engineering field. They’re quick, easy and inexpensive to grow, and their relatively simple biology provides the perfect sandbox for experimentation.
This is precisely the point where Shmuel Gliezer in Prof. Ron Milo’s lab at the Weissman Institute of Science started. Gliezer and colleagues set out to convert normal sugar-eating, heterotrophic E. coli, into a new autotrophic strain that generates all of its biomass from atmospheric CO2.
The next, undoubtedly bigger, obstacle is figuring out how to enable E. coli to fix atmospheric carbon into organic compounds. Milo looked to nature for inspiration. The most prevalent natural carbon fixation pathway, that dominates in plants, is the Calvin cycle; a process of 13 reactions revolving around a vital carbon-fixing enzyme called RuBisCO.
Milo’s lab implanted key elements of the Calvin cycle into their E. coli and synchronised the alien process with E. coli’s natural metabolism. They then further engineered their E. coli to utilise an electrochemically produceable compound called formate, that can provide the necessary reducing power to propel CO2 through the Calvin cycle reactions.
With all the pieces in hand, Milo’s lab set about trying to get them to fit together. In 2019, they achieved this major milestone. Their new, formate-powered, Calvin-cycle-containing, autotrophic E. coli was brought to life through “adaptive laboratory evolution”. This method involves employing the principles of natural selection in a laboratory environment and allowed Milo’s lab to successfully nudge E. coli towards accepting their new, sugar-free diet.
The powerful “adaptive laboratory evolution” technique involves generating various selection pressures that effectively provoke E. coli into adapting. Mutations that shift E. coli towards autotrophism are selected for again and again and, eventually, fully autotrophic E. coli is born.
One downside to this process is the accumulation of mutations whose function, or contribution to the autotrophic state, are not well understood. Not happy with such a black box, Milo’s lab dug deeper into the mutations enabling E. coli’s new autotrophic metabolism. Their recent paper revealed that, in fact, just three mutations are essential. Plugging these three mutations into E. coli, alongside the Calvin cycle and formate-utilisation modules, was sufficient to facilitate autotrophy.
Such an incredibly minimal system showcases the power of E. coli, and, importantly, expands our understanding of precisely what is needed to enable autotrophic growth, opening doors for future innovation.
At the Weissman Institute, Milo’s lab created their new organism by implanting nature’s tried and tested Calvin cycle into a surprisingly obliging E. coli. Meanwhile, a few thousand miles away at the Max Planck Institute for Terrestrial Microbiology, Tobias Erb’s lab was taking a different approach to making a new carbon-consuming creature.
Rather than use a pre-existing carbon-fixation pathway, Erb’s lab decided to create a new one. The idea may be conceptually simple but, considering that the most prevalent carbon-fixation pathway in nature is the result of billions of years of evolution, improving on this in the lab is a daunting feat.
Nonetheless, Erb’s lab got stuck in. The central enzyme of the Calvin cycle, RuBisCO, is notoriously inefficient, and so represented an obvious starting point for metabolic enhancement. Erb’s lab settled on phosphoenolpyruvate carboxylate and crotonyl-CoA carboxylase/reductase as RuBisCO replacements; two of the most efficient carbon-fixation enzymes in nature. They then designed a metabolic programme linking these enzymes together, to form a continuous, thermodynamically and biologically feasible cycle.
The result: the THETA cycle. A complex series of 17 reactions with acetyl-CoA (a biochemically useful compound) as the end-product.
Machine-learning-led optimisation of the THETA cycle led to several iterations with improved carbon-fixation rates, and a variety of useful output compounds, demonstrating the flexibility of the system.
Finally, Erb’s lab tried implementing their synthetic carbon-fixing pathway in a real, living organism. They took a piecemeal approach, dividing the cycle into 3 modules, and implementing each module separately. At each stage, coordinating E. coli’s natural metabolism with the THETA cycle represented walking a tight-rope, balancing side-reactions, by-products, and enzyme-bottlenecks in order to achieve harmony.
Realising the whole cycle at once remains to be seen, however, managing to bring each separate module of the synthetic THETA cycle to life in E. coli represents an important proof-of-concept. If scientists can dream it, the THETA-cycle success story suggests that perhaps they can do it too.
While both Milo’s and Erb’s research groups chose the well-known starting point of E. coli to engineer their new autotrophic organisms, C3 Biotechnologies have taken a different approach: discovering novel species with desirable growth traits.
C3’s goal is the biomanufacturing of industrially useful chemicals, including bio-propane and bio-plastics. A major obstacle in current bio-production is the high cost of microbial fermentations. Setting up microbial fermentations with optimal growth parameters for the microbes we currently rely on can be prohibitively expensive, for example requiring large quantities of fresh water. One possible solution to this is finding microbes that can thrive in extreme conditions (such as in waste-water or sea water).
Rather than engineering a new organism from scratch, C3 set out to discover if any such species was already out there. By screening environmental samples, C3 discovered a species of Halomonas bacteria they named Halomonas rowanensis. This isolate was found to be highly tolerant to salinity, pH, and to chemical precursors of their desired bio-propane end-product. Moreover, H. rowanensis was also found to be capable of autotrophic growth, utilising energy from thiosulfate to fix atmospheric carbon.
Theoretically, such a highly tolerant, autotrophic bacteria could be used for producing industrially useful bio-propane under non-sterile conditions, while using atmospheric CO2 as fuel.
C3 rapidly set out to prove this concept. By engineering H. rowanensis to express a specific enzyme variant that catalyses the decarboxylation of butyric acid to propane, C3 demonstrated that their novel H. rowanensis can produce bio-propane, as well as other industrially useful chemicals, from a diet of thiosulfate and CO2.
As well as the significant cost savings H. rowanensis could provide to bio-production processes due to requiring less-stringent growth parameters, the possibility of autotrophic growth could also significantly reduce the carbon footprint of these processes. In addition, many industries create waste-water that is contaminated with thiosulfate. Such waste-water could be captured and fed into H. rowanensis fermentation, for the dual purpose of bioremediation and bio-propane production.
The applications and potential of these new carbon-crunching bugs seems expansive. Perhaps the future holds sci-fi-esque bacteria hoovering up CO2 from our over-saturated atmosphere? In the more immediate term, these new bacteria could allow us switch industrial cell cultures from corn-syrup to a greener diet of electrochemically generated formate, or thiosulfate-contaminated waste-water, and air.
Either way, SynBio is just at the start of a potential engineered-organism revolution. With such major milestones already in the rearview, and with such clear industrial applications, we’ll certainly be keeping a close eye on this space.
Amelia is a trainee patent attorney in our life sciences team. Amelia has an undergraduate BSc degree in Biochemistry from Cardiff University with an Industrial placement at GlaxoSmithKline and a PhD Cancer Sciences from University of Manchester.
Email: amelia.jones@mewburn.com
Our IP specialists work at all stage of the IP life cycle and provide strategic advice about patent, trade mark and registered designs, as well as any IP-related disputes and legal and commercial requirements.
Our peopleWe have an easily-accessible office in central London, as well as a number of regional offices throughout the UK and an office in Munich, Germany. We’d love to hear from you, so please get in touch.
Get in touch