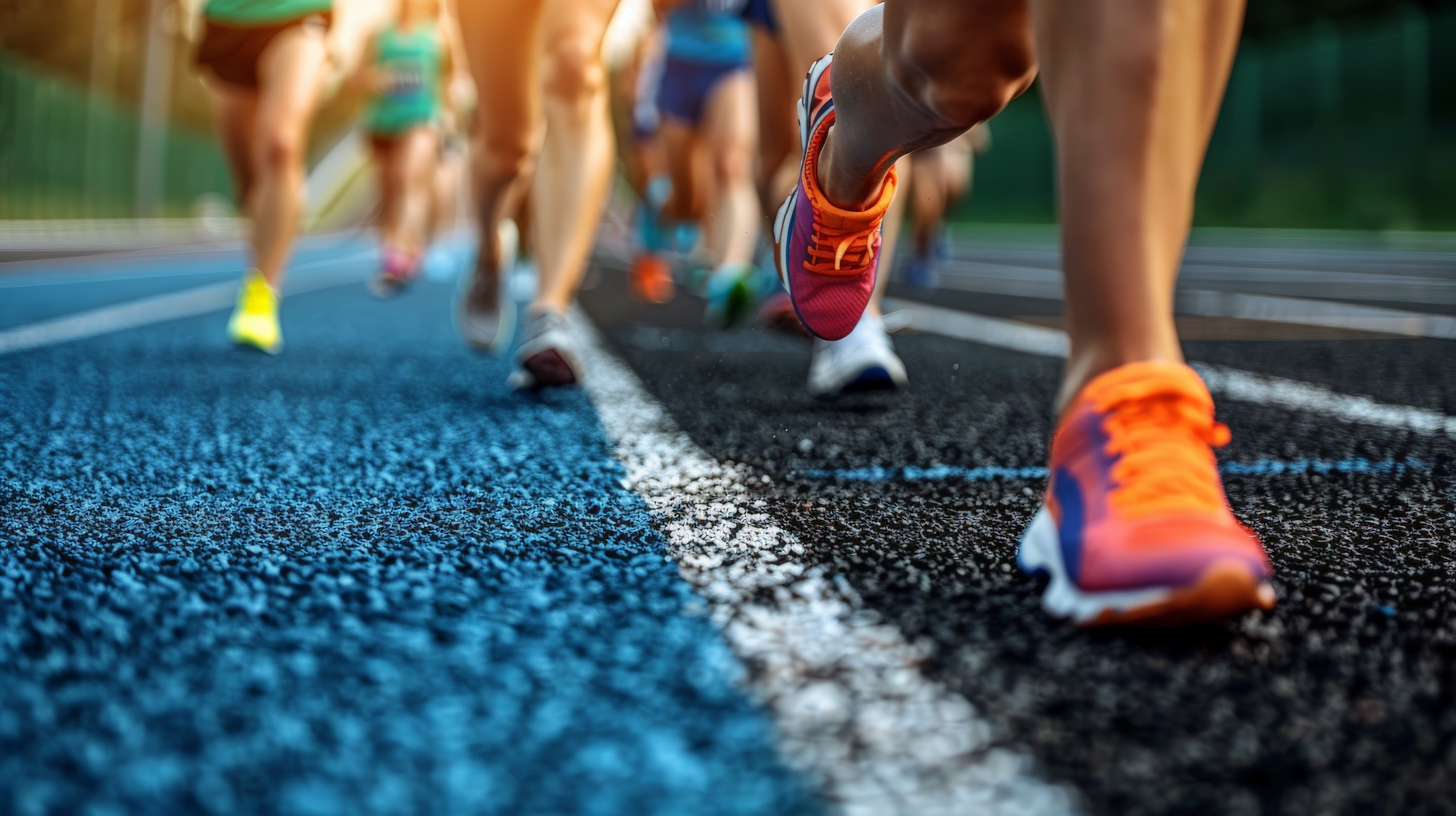
In 2023, gene therapy hit the headlines in the UK, when the BBC reported the cure of a toddler with metachromatic leukodystrophy (MLD) with the gene therapy drug Libmeldy. MLD is an inheritable lysosomal storage disease caused by a deficiency in the enzyme ARSA, which results in neurodegeneration. Previously MLD was a fatal condition, but Libmeldy delivers a working copy of the ARSA gene to the patient, rectifying the deficiency. If delivered before symptoms begin it will hopefully enable sufferers to lead normal, healthy lives.
Such stories have brought the transformative potential of gene therapy into public consciousness. Although first touted decades ago as a way to cure untreatable genetic conditions, it is only recently that the technology has begun to bear fruit, with the majority of gene therapies on the market having been approved since 2020.
In a series of articles, we will be looking at gene therapy, the technologies underlying it and its future directions. For those developing gene therapies, protection of their technology can be key to their ultimate commercial success, so we will also consider how intellectual property relating to gene therapy can be protected. Here at Mewburn Ellis we’re proud to work with our many clients at the forefront of this field of medicine.
Broadly speaking, gene therapy can be defined as any therapy which involves the manipulation of a patient’s genetic information. Gene therapy can be divided into two main types: ex vivo gene therapy, where a patient’s cells are removed from their body, modified and then reintroduced; and in vivo gene therapy, where genetic material is administered directly to a patient to modify cells within the body. Much of the initial focus of gene therapy was on the treatment of monogenic diseases (diseases caused by a deficiency in a single gene), where treatment could be effected by replacement or addition of the absent/faulty gene. However, the field has now evolved to consider any modification of gene expression which may be beneficial to a patient. Moreover, the development of gene editing techniques (e.g. CRISPR) has enabled a much wider array of possible genetic modifications.
One of the most promising treatment modalities for monogenic diseases involves ex vivo gene therapy using modified haematopoietic stem/progenitor cells (HSPCs)i, which can be isolated from a person’s blood. Autologous cells (derived from the patient to be treated) are commonly used to avoid the danger of graft-versus-host disease (GvHD), which is associated with imperfectly matched donor cells.
Isolated HSPCs are modified by insertion of the gene which is missing or defective in the person to be treated. Modification is generally performed by transduction of the cells with a viral vector, such as a lentiviral vector, which integrates into the genome of the HSPCs and results in permanent insertion of the therapeutic gene.
Prior to readministration of the cells the patient is “conditioned”, meaning that their endogenous, diseased HSPC population is (at least partly) depleted, by chemotherapy, radiotherapy and/or immunotherapy, to make way for the modified cells. Modified HSPCs are then infused back into the patient, where they engraft into the bone marrow and begin to proliferate and differentiate into cells which express the therapeutic gene.
Ex vivo HSPC gene therapy is particularly suitable for treating blood and immune disorders (since blood and immune cells develop from HSPCs). The first ex vivo gene therapy approved in Europe was Strimvelis, for the treatment of severe combined immunodeficiency caused by adenosine deaminase deficiency (ADA-SCID). Strimvelis uses modified HSPCs to deliver a functional adenosine deaminase gene to patientsii.
Some other types of disease can also be treated by ex vivo HSPC gene therapy, particularly diseases relating to the central nervous system (CNS), including neurometabolic disorders resulting from deficiencies in lysosomal or peroxisomal enzymes (such as MLD). While cells of the CNS cannot be replaced with modified HSPCs, myeloid cells originating from HSPCs are able to penetrate and take up residency in the CNS. Enzymes secreted from myeloid cells can be taken up by neighbouring cells of the CNS, thereby correcting their deficiency. Libmeldy works in this wayiii. However, genetic disorders affecting other organs are generally not treatable by ex vivo gene therapy.
Another type of genetically modified cell therapy is cellular immunotherapy, in which immune cells are modified to express an antigen receptor which targets diseased cells. We have reviewed cellular immunotherapy elsewhere.
A significant disadvantage of ex vivo gene therapy is its high cost, due to the therapy being personalised to each patient. For example, Libmeldy has a list price of £2.8 million per treatment, comfortably the highest of any medicine available in the UK (although the National Health Service has negotiated a discount). Such prices inevitably limit the availability of these advanced therapies.
Whereas ex vivo gene therapy entails the genetic modification of cells outside a patient’s body, in in vivo gene therapy a patient’s cells are genetically modified inside their body. A gene therapy product is administered to the patient either systemically (e.g. in the blood) or into a specific target organ. Viral vectors are commonly used for in vivo gene delivery, though non-viral delivery vehicles such as lipid nanoparticles (LNPs) are used in some cases.
Whereas ex vivo genetic modification of cells generally uses viruses which integrate into the genome of transduced cells, this can be an undesirable trait for a virus that is administered into a patient’s body. Instead, the leading viral vector for in vivo gene delivery is currently the adeno-associated virus (AAV). Upon infecting a cell, the AAV genome forms a DNA episome: a stable, non-chromosomal structure which can reside long-term in human cellsiv, and therefore can have a lasting or even permanent effect on gene expression. Modified AAVs have been developed which cannot replicate, providing a safer vehicle for in vivo gene therapyv.
Several in vivo gene therapies have been approved to date. For instance, Luxturna is an AAV-delivered gene therapy for inherited eye diseases caused by a deficiency in the gene RPE65, including some instances of Leber congenital amaurosis (LCA) and retinitis pigmentosa. Luxturna uses an AAV vector to deliver a functional copy of the gene to retinal cells, which can fully restore sight in some patients.
Looking forward, in vivo gene therapy has wider potential utility than ex vivo gene therapy, since it is not limited to treatment of diseases which affect any particular type of cell or tissue. Instead, in vivo gene therapy can be used to target almost any cell in the body. Even when administered systemically, a particular tissue or even cell type can be targeted by using a delivery vehicle with a particular tissue tropism or by placing the therapeutic gene under the control of a tissue/cell-specific promoter or enhancervi.
In vivo gene therapy is also generally less expensive than ex vivo therapy, as the therapeutic can be manufactured as a single pharmaceutical product to be administered to all patients (rather than requiring a personalised manufacturing process for each). That said, in vivo gene therapies at present still involve considerable expense. Luxturna, for instance, has a list price of around £600,000 per patient. It is expected that as the field advances, and standard delivery platforms are developed which can be used for multiple therapies, the costs will begin to fall.
Despite its potential, in vivo gene therapy is also not without technical challenges. Environmental exposure to AAVs mean that many people have neutralising antibodies against (at least some serotypes of) the virus, which can prevent cell binding and gene delivery following systemic administration of an AAV-based therapy. Even in seronegative individuals, administration of an AAV-based gene therapy results in an immune response to the virus, which will prevent systemic re-administration of the AAV. Administration of AAV-based therapies in situ to target organs can avoid these difficulties, and work is ongoing to develop “stealth” AAVs which do not stimulate an immune response in humansvii. Another limitation of AAVs is that their genome is only 4.7 kb in size. This prevents delivery of large genes, or multiple genes, in a single AAV. Work is ongoing to develop means of delivering larger therapeutic sequences.
Gene editing techniques, particularly CRISPR, have opened a whole new avenue of gene therapy: rather than inserting additional genes for expression in target cells, gene editing components can be delivered which are programmed to make changes (e.g. correct mutations) in a patient’s DNA in a targeted manner. Correcting chromosomal copies of genes is advantageous as the genes are under control of their native promoters and other regulatory elements, meaning they should be expressed at the correct level. Exogenous genes delivered using a viral vector are generally controlled by non-native regulatory elements, meaning that ensuring gene expression at the correct level can be challenging.
Excitingly, the first gene therapy employing gene editing was approved in September 2023. Casgevy treats sickle cell disease and β-thalassaemia, both of which are caused by errors in the haemoglobin β-chain (β-globin), resulting in dysfunctional haemoglobin. Unusually, humans have two sets of haemoglobin genes, encoding foetal haemoglobin and adult haemoglobin. Expression of foetal haemoglobin is switched off shortly after birth, with adult haemoglobin taking over. In most cases, people with defective β-globin genes have functional foetal haemoglobin genes, and Casgevy works by switching these back on. This is achieved by knocking out the gene BCL11a, which encodes the repressor protein responsible for switching off foetal haemoglobin expression.
Casgevy is an ex vivo gene therapy, which uses a non-viral method to deliver the CRISPR machinery to HSPCs obtained from each patient. Gene editing therapies are not limited to ex vivo treatments though, and several in vivo gene editing therapies are in development. For example, Intellia’s NTLA-2001 is a systemic, in vivo CRISPR-based gene therapy for hereditary transthyretin amyloidosis (ATTR), a fatal neurodegenerative disease caused by mutation of the TTR gene, which encodes the thyroxine transport protein transthyretin. NTLA 2001 uses an LNP to deliver CRISPR components to the liver (where TTR is primarily synthesised), which knock out the TTR gene. A phase 3 trial of NTLA 2001 has recently been approved.
Patent protection is as important for gene therapies as for traditional pharmaceutical products, and patentable inventions can be found in many aspects of gene therapies. Where a gene therapy delivers a naturally occurring nucleic acid to a patient, that nucleic acid itself may not be patentable. However, this is in practice a rather narrow exclusion. For example, cDNA or codon-optimised sequences are patentable, as are expression cassettes, viral vectors or other delivery vehicles which contain therapeutic genes. Modified stem cells for ex vivo gene therapy are also patentable in most countries, so long as they are distinguishable from naturally occurring stem cells (thus stem cells with a therapeutic gene inserted, for example, are patentable, but stem cells in which a mutation has been corrected by gene editing may not be).
Platforms for gene therapy may also be patentable, for example viral vectors or nanoparticles which may be useful for a variety of therapies. Therapeutic methods, including gene therapies, are also patentable in many countries (in one form or another).
After decades of promise, the time of gene therapy may finally have arrived, with clinical advances seeming to occur with astonishing frequency. In the coming months, we’ll be publishing a series of articles considering particular technical, regulatory and IP considerations for gene therapy in more detail. If you want any advice relating to protecting a gene therapy technology, we’d love to hear from you.
Ed handles patent work across the life sciences/biotech field, with a particular focus on antibody therapeutics, cell therapy and vaccines. Outside of the medical sphere he also has extensive experience in enzyme technology. Ed is very experienced in drafting patent applications and prosecuting them around the world, and also handles opposition and appeal work at the European Patent Office.
Email: edward.couchman@mewburn.com
Our IP specialists work at all stage of the IP life cycle and provide strategic advice about patent, trade mark and registered designs, as well as any IP-related disputes and legal and commercial requirements.
Our peopleWe have an easily-accessible office in central London, as well as a number of regional offices throughout the UK and an office in Munich, Germany. We’d love to hear from you, so please get in touch.
Get in touch